甲烷转化
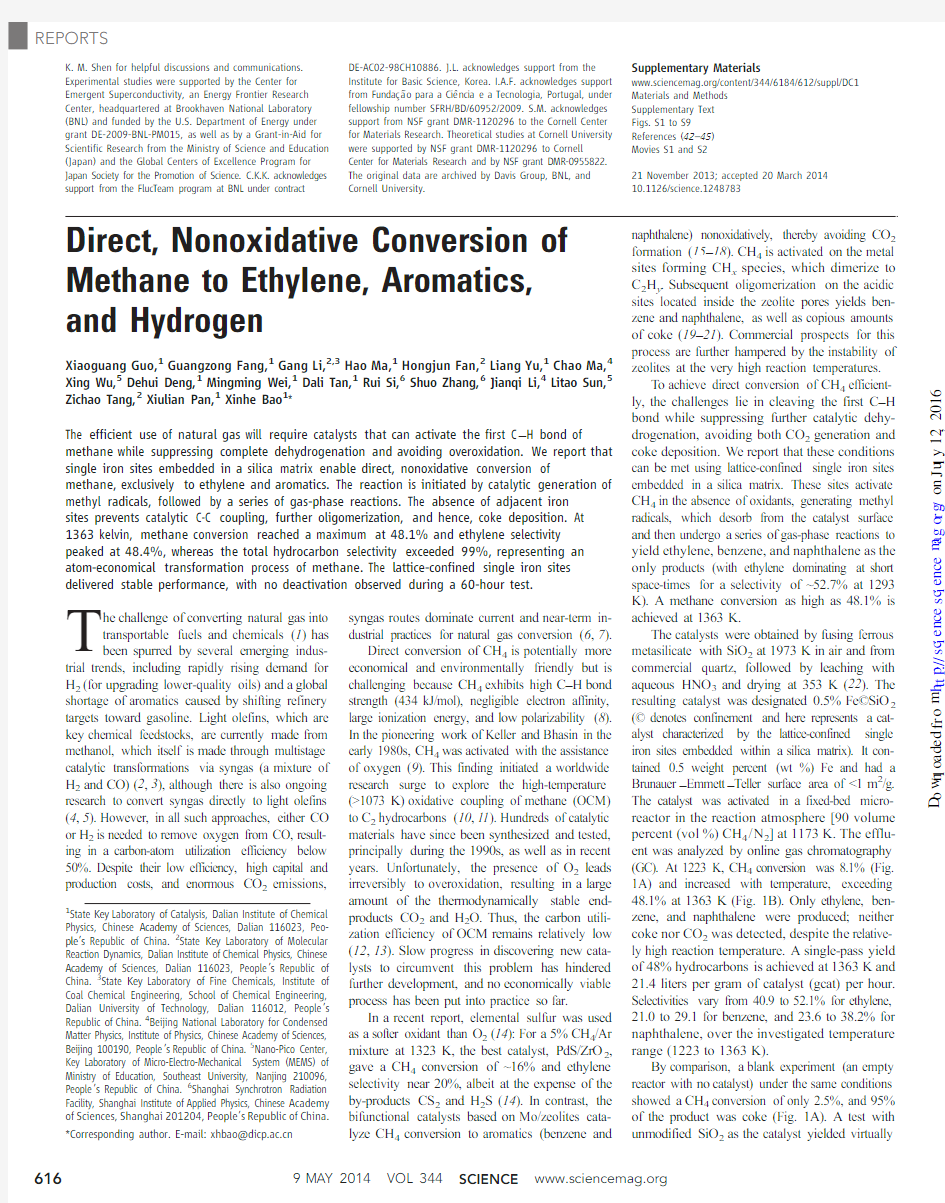

K.M.Shen for helpful discussions and communications.Experimental studies were supported by the Center for Emergent Superconductivity,an Energy Frontier Research Center,headquartered at Brookhaven National Laboratory (BNL)and funded by the U.S.Department of Energy under grant DE-2009-BNL-PM015,as well as by a Grant-in-Aid for Scientific Research from the Ministry of Science and Education (Japan)and the Global Centers of Excellence Program for Japan Society for the Promotion of Science.C.K.K.acknowledges support from the FlucTeam program at BNL under contract
DE-AC02-98CH10886.J.L.acknowledges support from the Institute for Basic Science,Korea.I.A.F.acknowledges support from Funda??o para a Ciência e a Tecnologia,Portugal,under fellowship number SFRH/BD/60952/2009.S.M.acknowledges support from NSF grant DMR-1120296to the Cornell Center for Materials Research.Theoretical studies at Cornell University were supported by NSF grant DMR-1120296to Cornell
Center for Materials Research and by NSF grant DMR-0955822.The original data are archived by Davis Group,BNL,and Cornell University.
Supplementary Materials
https://www.360docs.net/doc/8f4126875.html,/content/344/6184/612/suppl/DC1Materials and Methods Supplementary Text Figs.S1to S9
References (42–45)Movies S1and S2
21November 2013;accepted 20March 201410.1126/science.1248783
Direct,Nonoxidative Conversion of Methane to Ethylene,Aromatics,and Hydrogen
Xiaoguang Guo,1Guangzong Fang,1Gang Li,2,3Hao Ma,1Hongjun Fan,2Liang Yu,1Chao Ma,4Xing Wu,5Dehui Deng,1Mingming Wei,1Dali Tan,1Rui Si,6Shuo Zhang,6Jianqi Li,4Litao Sun,5Zichao Tang,2Xiulian Pan,1Xinhe Bao 1*
The efficient use of natural gas will require catalysts that can activate the first C –H bond of
methane while suppressing complete dehydrogenation and avoiding overoxidation.We report that single iron sites embedded in a silica matrix enable direct,nonoxidative conversion of
methane,exclusively to ethylene and aromatics.The reaction is initiated by catalytic generation of methyl radicals,followed by a series of gas-phase reactions.The absence of adjacent iron sites prevents catalytic C-C coupling,further oligomerization,and hence,coke deposition.At 1363kelvin,methane conversion reached a maximum at 48.1%and ethylene selectivity peaked at 48.4%,whereas the total hydrocarbon selectivity exceeded 99%,representing an atom-economical transformation process of methane.The lattice-confined single iron sites delivered stable performance,with no deactivation observed during a 60-hour test.T
he challenge of converting natural gas into transportable fuels and chemicals (1)has been spurred by several emerging indus-trial trends,including rapidly rising demand for H 2(for upgrading lower-quality oils)and a global shortage of aromatics caused by shifting refinery targets toward gasoline.Light olefins,which are key chemical feedstocks,are currently made from methanol,which itself is made through multistage catalytic transformations via syngas (a mixture of H 2and CO)(2,3),although there is also ongoing research to convert syngas directly to light olefins (4,5).However,in all such approaches,either CO or H 2is needed to remove oxygen from CO,result-ing in a carbon-atom utilization efficiency below 50%.Despite their low efficiency,high capital and production costs,and enormous CO 2emissions,
syngas routes dominate current and near-term in-dustrial practices for natural gas conversion (6,7).Direct conversion of CH 4is potentially more economical and environmentally friendly but is challenging because CH 4exhibits high C –H bond strength (434kJ/mol),negligible electron affinity,large ionization energy,and low polarizability (8).In the pioneering work of Keller and Bhasin in the early 1980s,CH 4was activated with the assistance of oxygen (9).This finding initiated a worldwide research surge to explore the high-temperature (>1073K)oxidative coupling of methane (OCM)to C 2hydrocarbons (10,11).Hundreds of catalytic materials have since been synthesized and tested,principally during the 1990s,as well as in recent years.Unfortunately,the presence of O 2leads irreversibly to overoxidation,resulting in a large amount of the thermodynamically stable end-products CO 2and H 2O.Thus,the carbon utili-zation efficiency of OCM remains relatively low (12,13).Slow progress in discovering new cata-lysts to circumvent this problem has hindered further development,and no economically viable process has been put into practice so far.
In a recent report,elemental sulfur was used as a softer oxidant than O 2(14):For a 5%CH 4/Ar mixture at 1323K,the best catalyst,PdS/ZrO 2,gave a CH 4conversion of ~16%and ethylene selectivity near 20%,albeit at the expense of the by-products CS 2and H 2S (14).In contrast,the bifunctional catalysts based on Mo/zeolites cata-lyze CH 4conversion to aromatics (benzene and
naphthalene)nonoxidatively,thereby avoiding CO 2formation (15–18).CH 4is activated on the metal sites forming CH x species,which dimerize to C 2H y .Subsequent oligomerization on the acidic sites located inside the zeolite pores yields ben-zene and naphthalene,as well as copious amounts of coke (19–21).Commercial prospects for this process are further hampered by the instability of zeolites at the very high reaction temperatures.To achieve direct conversion of CH 4efficient-ly,the challenges lie in cleaving the first C –H bond while suppressing further catalytic dehy-drogenation,avoiding both CO 2generation and coke deposition.We report that these conditions can be met using lattice-confined single iron sites embedded in a silica matrix.These sites activate CH 4in the absence of oxidants,generating methyl radicals,which desorb from the catalyst surface and then undergo a series of gas-phase reactions to yield ethylene,benzene,and naphthalene as the only products (with ethylene dominating at short space-times for a selectivity of ~52.7%at 1293K).A methane conversion as high as 48.1%is achieved at 1363K.
The catalysts were obtained by fusing ferrous metasilicate with SiO 2at 1973K in air and from commercial quartz,followed by leaching with aqueous HNO 3and drying at 353K (22).The resulting catalyst was designated 0.5%Fe?SiO 2(?denotes confinement and here represents a cat-alyst characterized by the lattice-confined single iron sites embedded within a silica matrix).It con-tained 0.5weight percent (wt %)Fe and had a Brunauer –Emmett –Teller surface area of <1m 2/g.The catalyst was activated in a fixed-bed micro-reactor in the reaction atmosphere [90volume percent (vol %)CH 4/N 2]at 1173K.The efflu-ent was analyzed by online gas chromatography (GC).At 1223K,CH 4conversion was 8.1%(Fig.1A)and increased with temperature,exceeding 48.1%at 1363K (Fig.1B).Only ethylene,ben-zene,and naphthalene were produced;neither coke nor CO 2was detected,despite the relative-ly high reaction temperature.A single-pass yield of 48%hydrocarbons is achieved at 1363K and 21.4liters per gram of catalyst (gcat)per hour.Selectivities vary from 40.9to 52.1%for ethylene,21.0to 29.1for benzene,and 23.6to 38.2%for naphthalene,over the investigated temperature range (1223to 1363K).
By comparison,a blank experiment (an empty reactor with no catalyst)under the same conditions showed a CH 4conversion of only 2.5%,and 95%of the product was coke (Fig.1A).A test with unmodified SiO 2as the catalyst yielded virtually
1
State Key Laboratory of Catalysis,Dalian Institute of Chemical Physics,Chinese Academy of Sciences,Dalian 116023,Peo-ple ’s Republic of China.2State Key Laboratory of Molecular Reaction Dynamics,Dalian Institute of Chemical Physics,Chinese Academy of Sciences,Dalian 116023,People ’s Republic of China.3State Key Laboratory of Fine Chemicals,Institute of Coal Chemical Engineering,School of Chemical Engineering,Dalian University of Technology,Dalian 116012,People ’s Republic of China.4Beijing National Laboratory for Condensed Matter Physics,Institute of Physics,Chinese Academy of Sciences,Beijing 100190,People ’s Republic of China.5Nano-Pico Center,Key Laboratory of Micro-Electro-Mechanical System (MEMS)of Ministry of Education,Southeast University,Nanjing 210096,People ’s Republic of China.6Shanghai Synchrotron Radiation Facility,Shanghai Institute of Applied Physics,Chinese Academy of Sciences,Shanghai 201204,People ’s Republic of China.*Corresponding author.E-mail:xhbao@https://www.360docs.net/doc/8f4126875.html,
9MAY 2014VOL 344
SCIENCE
https://www.360docs.net/doc/8f4126875.html,
616REPORTS
o n J u l y 12, 2016
h t t p ://s c i e n c e .s c i e n c e m a g .o r g /D o w n l o a d e d f r o m
the same result (table S1)(22).Most notably,the use of 0.5wt %Fe/SiO 2as the catalyst (prepared with wet impregnation on amorphous SiO 2with a high surface area,348m 2/g)(22)also led to high coke formation (>98%).We have varied the iron loadings,support materials,and preparation methods,which unfortunately do not preclude coke on iron nanoparticles (NPs).For example,coke remained the principal product (>50%)over 0.5%Fe/ZSM.0.8%Fe/SiO 2prepared by sol-gel method and 0.2%Fe/SiC (22)enhanced selective formation of hydrocarbons to some extent,but still with a considerable amount of coke (Fig.1A).Figure S1(22)demonstrates that the reac-tivity of 0.5%Fe?SiO 2was very reproducible.All mass balances are above 99%.At 1363K,the space-time yields for ethylene,benzene,and naphthalene were 91,18,and 9mol kgcat –1hour –1,respectively.Moreover,the process represents a new and sustainable approach to H 2production because the by-products are high –value-added hydrocarbons (ethylene and aromatics)instead of coke and CO 2(23,24).The yield of H 2varies with the reaction conditions,and the concentra-tions in the effluent range from 10.9to 51.2%(fig.1D)(22).
Furthermore,the 0.5%Fe?SiO 2catalyst was very stable,and no deactivation was observed
during a 60-hour test at 1293K (Fig.1C).Meth-ane conversion remained at ~32%throughout this long run.Selectivities to ethylene (52.7%),benzene (21.5%),and naphthalene (25.8%)were constant,and the total selectivity to these pro-ducts remained >99%.The combination of atom-economy,high selectivity,and high conversion is notable,considering the rather low loading of Fe (0.5wt %)and very low surface area (<1m 2/g)of the SiO 2.Although noncatalytic pyrolysis of CH 4has been extensively studied for light hydrocarbon synthesis,the product is dominated with acetylene accompanied by high coke formation (25–27).By comparison,the catalytically initiated reaction de-scribed here compares very favorably with other reported direct-conversion processes,including pyrolysis,OCM (13),and nonoxidative aromati-zation (21).Finally,because natural gas usually contains some ethane,we added 1and 5vol %ethane to the reactant stream.The presence of ethane substantially enhanced methane conver-sion (fig.S2)(22),and ethane is almost completely converted,although a small amount of coke is formed at 1173K and a space velocity of 4.84liter gcat –1h –1.
The unprecedented efficiency of the catalyt-ically initiated CH 4conversion process is attri-buted to the high activity of the coordinatively
unsaturated iron sites toward the C-H bond of CH 4(28,29).The isolated nature of these sites,as evidenced by sub-angstrom –resolution high-angle annular-dark field (HAADF)scanning transmis-sion electron microscopy (STEM)and in situ x-ray absorption near-edge spectroscopy (XANES),pre-cludes surface C-C coupling and,hence,coke formation.Transmission electron microscopy in-dicates that in the fresh 0.5%Fe?SiO 2catalyst,iron oxide NPs with a size of ~3to 4nm are distributed homogeneously throughout the SiO 2matrix (fig.S3)(22).A STEM-HAADF image of the catalyst after reaction reveals many bright dots of atomic size scattered across the SiO 2matrix,highlighted by the red circles in Fig.2A.Each dot represents an individual Fe atom,con-sidering the much lower contrast of Si and O in the HAADF image.This result suggests that the iron species are redistributed from the original oxide NPs to isolated atoms during catalyst activation.This hypothesis is validated by the in situ XANES during activation.The near-edge spec-trum of the catalyst is similar to that of Fe foil (Fig.2B).In Fig.2C,the Fourier-transformed k 3-weighted c (k )function (where k is wave number)(30)shows that,after activation,the Fe-O scattering paths apparent in the spectrum of the fresh catalyst (line 1)have
disappeared,
Fig.1.Reaction performance.(A )Comparison of different catalysts at 1223K and 4.84liter gcat –1hour –1.(B )Effect of reaction temperatures and space velocities on the 0.5%Fe?SiO 2catalyst.Blue circles denote CH 4con-version,whereas bars represent product selectivities.(C )Long-term stability
test of 0.5%Fe?SiO 2at 1293K and 14.5liter gcat –1hour –1.(D )(Top)Hydrogen contents of the reactor effluent (open circles)and the calculated values (solid circles);(bottom)H 2peaks in GC analysis normalized by the internal standard N 2(22).a.u.,arbitrary units.
https://www.360docs.net/doc/8f4126875.html,
SCIENCE VOL 344
9MAY 2014
617
REPORTS
o n J u l y 12, 2016
h t t p ://s c i e n c e .s c i e n c e m a g .o r g /D o w n l o a d e d f r o m
whereas new scattering paths appear (line 2).They are assigned to Fe-C and Fe-Si paths,by comparison to the spectra of reference materials such as Fe 2O 3,FeSi 2,and iron carbides (31).In the presence of CH 4above 1173K,iron oxide species in the fresh 0.5%Fe?SiO 2interact ex-tensively with the support,becoming embed-ded in the silica matrix through bonding to Si and C atoms.Thus,these otherwise extremely reactive,coordinatively unsaturated iron atoms are stabilized and persist under the very harsh reaction conditions.No aggregation was observed,even after prolonged reaction for 60hours.
In contrast,the 2-to 5-nm-sized iron NPs in 0.5%Fe/SiO 2(fig.S5a)(22)after activation un-der the same conditions exhibit only a Fe –Fe bond (line 3in Fig.2,B and C).This result explains the extensive carbon deposition observed for 0.5%Fe/SiO 2,considering that iron NPs are widely used for the synthesis of carbon nanotubes (32).That process involves catalytic cleavage of C –H bonds and dissolution of carbon species into the iron lattice.Subsequent C-C coupling on an iron NP surface and crystallization from the super-saturated carbide solid solution drive the growth of nanotubes (33).However,under the harsh re-action conditions in the current reaction,0.5%Fe/SiO 2deactivates very rapidly,and iron NPs aggregated and grew to 20to 30nm after reaction (fig.S4b)(22).These results again highlight the crucial role played by the site isolation of the iron species in 0.5%Fe?SiO 2in achieving high sel-ectivity toward hydrocarbons and preventing coke formation.
Furthermore,density functional theory (DFT)calculations suggest that the most stable struc-ture in the reactive atmosphere is an iron atom coordinated by one Si and two C atoms and is thus embedded within the SiO 2matrix,as de-picted in Fig.3A and fig.S5.The calculated Fe –C and Fe –Si bond lengths are 1.6and 2.4?,respec-tively,which are consistent with those estimated from extended x-ray absorption fine structure (EXAFS)(table S2)(22).This lattice-confined single iron site initiates CH 4dehydrogenation by generating a ?CH 3radical,which subsequently releases from the surface with an energy barrier of 2.32eV instead of undergoing further dehydro-genation or C-C coupling (fig.S5)(22).The Fe site is then exposed and becomes active for adsorp-tion of a second methane molecule and release of another methyl radical,with energy barriers of 3.07and 2.19eV ,respectively (Fig.3A).Migra-tion of H from C in Fe-C-Si sites to Fe involves a barrier of 0.58eV .The resulting surface H species desorbs as H 2with an energy barrier of 1.61eV .The intermediacy of methyl radicals was verified by online vacuum ultraviolet soft photoioni-zation molecular-beam mass spectrometry (VUV-SPI-MBMS)(fig.S6)(22).Molecules were ionized with a 10.6-eV VUV lamp,which has an energy lower than the CH 4ionization energy (12.6eV).This allows detection of intermediate radicals and products (34,35).Figure 3B and its inset display all species detected at 1193K.Methyl radicals,
represented by the signal at mass/charge ratio (m /z )=15,are clearly observed.Additional sig-nals at m /z =28,40,42,78,92,and 128are assigned to ethylene (C 2H 4),propyne or propadiene (C 3H 4),propylene (C 3H 6),benzene (C 6H 6),toluene (C 7H 8),and naphthalene (C 10H 8),respectively.To further elucidate the mechanism,the reac-tion profile of methyl radicals at 1225K was sim-ulated with DFT (Fig.3C and fig.S7)(22).Two ?CH 3radicals combine to form C 2H 6via a strongly exothermic process.C 2H 6undergoes dehydrogen-ation readily,giving C 2H 4and H atoms with an energy barrier of 1.58eV .By abstraction of H from C 2H 4,the resulting ?C 2H 3radical tends to react with additional C 2H 4molecules.Further de-hydrogenation and cyclization leads to benzene,with an energy barrier of 2.85eV .C 6H 6is also readily dehydrogenated by ?H and,after further chain growth and cyclization,yields the thermody-namically more stable naphthalene.The low bar-rier for transformation of C 2H 6to C 2H 4explains the absence of C 2H 6among the experimentally observed products under steady-state reaction con-ditions,whereas the thermodynamically more stable hydrocarbons C 2H 4,C 6H 6,and C 10H 8ac-cumulated and were detected.
At equilibrium at 1225K and atmospheric pressure,the yields of C 2H 4,C 6H 6,and C 10H 8from CH 4were estimated to be 9.0,34.0,and 57.0%(22),respectively.The relative ratios of these products could be manipulated by changing the reaction conditions.For example,increasing the CH 4flow rate in the VUV-SPI-MBMS re-actor favors formation of C 2H 4(Fig.3D),whereas lower flow rates (corresponding to longer resi-dence times)promote cyclization of intermediates leading to aromatics,which are consistent with the GC analysis obtained in the microreactor.These results lend further support to the hypothesis that the reaction is initiated by the catalytic generation of methyl radicals,which subsequently undergo a series of gas-phase reactions.Thus,the conversion efficiency is high,despite the very limited number of surface iron sites and the extremely low surface area of the catalyst.
Heterogeneous systems for CH 4activation gen-erally still suffer from poor carbon utilization,caused in part by low selectivity.Here,we dem-onstrate an atom-economical direct CH 4conver-sion process,enabled by the lattice-confined single iron sites embedded within a silica matrix,which activate CH 4and generate methyl radicals.A conversion as high as 48.1%was obtained at 1363K and a space velocity of 21.4liter gcat –1hour –1,with a selectivity to C 2H 4of >48.4%(the re-mainder being aromatics).No deactivation was observed even after reaction for 60hours,and the total carbon selectivity to the three products re-mained >99%.Although the dehydrogenation itself is endothermic,high selectivity to ethylene in this process substantially reduces the heat input (estimated to be about half that of a typical thermal pyrolysis process with dominating acet-ylene in product),as shown in table S3(22).These findings open up new possibilities for fundamental studies of direct,nonoxidative activation of CH 4
.
Fig.2.Structural features of 0.5%Fe?SiO 2.(A )STEM-HAADF image of the catalyst after reaction,with the inset showing the computational model of the single iron atom bonded to two C atoms and one Si atom within silica matrix.(B )In situ XANES upon activation and (C )Fourier transformed (FT)k 3-weighted c (k )-function of the EXAFS spectra.Solid lines denote reference samples of Fe foil,FeSi 2,and Fe 2O 3.Line 1denotes the fresh 0.5%Fe?SiO 2.Line 2stands for 0.5%Fe?SiO 2and line 3for 0.5%Fe/SiO 2upon activation in 10%CH 4/N 2at 1173K for 2hours,respectively.R(?),distance in angstroms.9MAY 2014VOL 344
SCIENCE
https://www.360docs.net/doc/8f4126875.html,
618REPORTS
o n J u l y 12, 2016
h t t p ://s c i e n c e .s c i e n c e m a g .o r g /D o w n l o a d e d f r o m
It is anticipated that combining a catalyst such as this one with an efficient reactor technology may enable the development of non –syngas-based routes to transform light hydrocarbons into high –value-added chemicals.
References and Notes
https://www.360docs.net/doc/8f4126875.html,/lab/ACC-US-chemical-investment-linked-to-shale-gas-reaches-$100-billion_58946.html.
2.J.Li et al .,J.Am.Chem.Soc.134,836–839(2012).
3.F.Diederich,Angew.Chem.Int.Ed.52,6–7(2013).
4.H.M.T.Galvis et al .,J.Am.Chem.Soc.134,16207–16215(2012).
5.H.M.Torres Galvis et al .,Science 335,835–838(2012).
6.Note that the construction of two megascale methanol plants in the U.S.Pacific Northwest was recently
announced to supply olefin feedstocks to Dalian,https://www.360docs.net/doc/8f4126875.html,/lab/Chinese-group-plans-two-mega-methanol-plants-in-USPacific-Northwest-to-supply-olefins-feedstock_58289.html.
https://www.360docs.net/doc/8f4126875.html,rkins,A.Z.Khan,Aust.J.Chem.42,1655–1670(1989).
9.G.E.Keller,M.M.Bhasin,J.Catal.73,9–19(1982).10.H.Arakawa et al .,Chem.Rev.101,953–996(2001).11.J.H.Lunsford,Angew.Chem.Int.Ed.Engl.34,970–980
(1995).
12.S.Arndt et al .,Catal.Rev.Sci.Eng.53,424–514(2011).
13.U.Zavyalova,M.Holena,R.Schl?gl,M.Baerns,
ChemCatChem 3,1935–1947(2011).
14.Q.Zhu et al .,Nat.Chem.5,104–109(2013).15.L.S.Wang et al .,Catal.Lett.21,35–41(1993).16.B.M.Weckhuysen,D.J.Wang,M.P.Rosynek,
J.H.Lunsford,J.Catal.175,338–346(1998).17.R.W.Borry,Y.H.Kim,A.Huffsmith,J.A.Reimer,
E.Iglesia,J.Phys.Chem.B 103,5787–5796(1999).18.D.J.Wang,J.H.Lunsford,M.P.Rosynek,J.Catal.169,
347–358(1997).
19.D.Ma et al .,J.Catal.208,260–269(2002).
20.R.Ohnishi,S.T.Liu,Q.Dong,L.Wang,M.Ichikawa,
J.Catal.182,92–103(1999).
21.S.Ma,X.Guo,L.Zhao,S.Scott,X.Bao,J.Energy Chem.
22,1–20(2013).
22.Supplementary materials are available on Science Online.23.T.V.Choudhary,C.Sivadinarayana,C.C.Chusuei,
A.Klinghoffer,D.W.Goodman,J.Catal.199,9–18(2001).24.M.A.Ermakova,D.Y.Ermakov,A.L.Chuvilin,
G.G.Kuvshinov,J.Catal.201,183–197(2001).25.A.Beloqui Redondo,E.Troussard,J.A.van Bokhoven,
Fuel Process.Technol.104,265–270(2012).26.A.Holmen,O.Olsvik,O.A.Rokstad,Fuel Process.
Technol.42,249–267(1995).
27.G.P.Van Der Zwet,P.A.J.M.Hendriks,R.A.Van Santen,
Catal.Today 4,365–369(1989).
28.H.Schwarz,Angew.Chem.Int.Ed.50,10096–10115
(2011).
29.E.W.McFarland,H.Metiu,Chem.Rev.113,4391–4427
(2013).
30.B.Qiao et al .,Nat.Chem.3,634–641(2011).
31.E.de Smit,A.M.Beale,S.Nikitenko,B.M.Weckhuysen,
J.Catal.262,244–256(2009).
32.R.C.Che,L.M.Peng,X.F.Duan,Q.Chen,X.L.Liang,
Adv.Mater.16,401–405(2004).
33.X.Pan et al .,Nat.Mater.6,507–511(2007).34.Z.Zhou,H.Guo,F.Qi,Trends Analyt.Chem.30,
1400–1409(2011).
35.L.Luo et al .,Sci.Rep.3,1625(2013).
Acknowledgments:This work was financially supported by the “Strategic Priority Research Program ”of the Chinese Academy of Sciences (grant XDA09030101),the National Natural Science Foundation of China (grants 21321002,11079005,21033009,and 21103181),and the Ministry of Science and Technology of China (grants 2011CBA00503and 2013CB933100).We thank S.L.Scott and H.Metiu for fruitful discussion.An international patent application under the Patent Cooperation Treaty is pending (PCT/CN2013/079977).
Supplementary Materials
https://www.360docs.net/doc/8f4126875.html,/content/344/6184/616/suppl/DC1Materials and Methods Figs.S1to S7Tables S1to S3References (36–66)
10March 2014;accepted 15April 2014
10.1126/science.1253150
Fig.3.Investigation of the reaction mechanism over 0.5%Fe?SiO 2.(A )DFT calculations on catalytic generation of methyl radicals at 1223K.(B )Species in the reactor effluent at 1193K,detected by VUV-SPI-MBMS.amu,atomic mass units.(C )DFT simulated reaction profile of methyl radicals in the gas phase at 1225K.D G,Gibbs free energy.(D )Relative intensity of VUV-SPI-MBMS signals of major products as a function of CH 4flow rate at 1223K.
https://www.360docs.net/doc/8f4126875.html, SCIENCE VOL 3449MAY 2014619
REPORTS
o n J u l y 12, 2016
h t t p ://s c i e n c e .s c i e n c e m a g .o r g /D o w n l o a d e d f r o m
(6184), 616-619. [doi: 10.1126/science.1253150]
344Science Pan and Xinhe Bao (May 8, 2014)
Tan, Rui Si, Shuo Zhang, Jianqi Li, Litao Sun, Zichao Tang, Xiulian Liang Yu, Chao Ma, Xing Wu, Dehui Deng, Mingming Wei, Dali Xiaoguang Guo, Guangzong Fang, Gang Li, Hao Ma, Hongjun Fan,Aromatics, and Hydrogen
Direct, Nonoxidative Conversion of Methane to Ethylene,
Editor's Summary
avoided surface reactions between the radicals that would deposit solid carbon.in the gas phase to form ethylene and aromatics along with hydrogen. The isolation of the active sites exposes methane to isolated iron sites on a silica catalyst. Methyl radicals were generated and coupled (p. 616) report a high-temperature nonoxidative route that et al.Guo tend to overoxidize the products. provide chemical feedstocks. However, the reaction conditions needed to activate the strong C-H bond Direct routes to converting methane to higher hydrocarbons can allow natural gas to be used to Upgrading Methane Sans Oxygen
This copy is for your personal, non-commercial use only.
Article Tools
https://www.360docs.net/doc/8f4126875.html,/content/344/6184/616article tools:
Visit the online version of this article to access the personalization and Permissions
https://www.360docs.net/doc/8f4126875.html,/about/permissions.dtl
Obtain information about reproducing this article: is a registered trademark of AAAS.
Science Advancement of Science; all rights reserved. The title Avenue NW, Washington, DC 20005. Copyright 2016 by the American Association for the
in December, by the American Association for the Advancement of Science, 1200 New York (print ISSN 0036-8075; online ISSN 1095-9203) is published weekly, except the last week Science o n J u l y 12, 2016
h t t p ://s c i e n c e .s c i e n c e m a g .o r g /D o w n l o a d e d f r o m
甲烷水蒸汽转化
天然气转化
天然气转化 甲烷水蒸汽转化(sMR) 甲烷水蒸汽转化工艺(SMR)作为传统的甲烷制合成气过程(图1一2),主要涉及下述反应: CH4+H2O!3H2+COvH298K=206.29kJ/mol 这是一个强吸热过程,转化一般要在高温下进行(>1073K)"产物中HZ/Co约为3:1,为防止催化剂积炭,通常需要通入过量的水蒸汽,依合成气用途,原料气 中HZO/CH4典型的摩尔比为2-5;并且为保持较高的生产速率,工业生产中压力通常高3.OMPa。该反应过程的缺点是能耗高,设备庞大复杂!占地面积大,投资和操作费用昂贵。 联合转化工艺(SM侧oZR) 联合重整工艺流程如图1-3所示,将SMR反应器出口的混合气送入二级氧化反应器内,未完全消耗的甲烷(在SMR出口处CH;转化率为90-92%)与0:发生部分
氧化反应后,再进一步通过催化剂床层进行二次重整反应,生成的合成气HZ/CO 比在2.5~4.0,随后利用水汽转化(WGS)反应(见式4),调整产品中H:和CO比例,来满足下游合成的利用。该工艺有效地减小了SRM的规模,降低了能耗,但不足之处是仍需两个反应器。 CH4+HZO03H2+COvH29sK=一4IkJ/mol 中国石化集团四川维尼纶厂目前在运行的甲醇装置有两套,一为1996年建成投产的直接以天然气为原料的10万t/a甲醇装置,另一为2011年整合建成投产的以乙炔尾气为原料的77万t/a甲醇装置。前者采用成熟的管式转化炉生产合成气,并利用德国Lurgi合成工艺技术生产甲醇;后者利用英国Davy公司合成工艺生产甲醇,并在合成环路驰放气的处理上采用了膜分离与ATR 转化工艺技术,以提高装置产能和降低综合能耗。10万t/a甲醇装置通过天然气蒸汽转化制取合成气,故合成气具有氢多、碳少、惰性气体(CH4、N2、Ar 等)含量低的特点,其气质组成有利于甲醇合成反应。77万t/a 甲醇装置以乙炔尾气为原料,由于乙炔尾气属于天然气部分氧化法制乙炔工艺的副产气,因而具有氢少、碳多、惰性气体含量偏高的特点,属于乏氢气质,需对系统进行补氢。为深度利用甲醇合成环路驰放气和提高装置产能,工艺上增设了膜分离与ATR转化流程,但伴随而来的是驰放气中大量惰性气体随 ATR 转化气循环返回合成系统并累积,导致合成环路惰性气体的体积分数长期高达25%~30%,这也是该套装置甲醇产品质量不易控制、部分物耗能耗指标达不到设计值且制约甲醇产量进一步提高的主要原因。针对如何利用天然气制合成气来降低乙炔尾气甲醇装置合成环路的惰性气体含量,提高甲醇产量,使装置运行更加优化与合理,本文通过现场调查以及对相关数据的计算、分析和研究,提出可工程实施的优化运行方案。
甲烷转化的基础知识
甲烷转化的基础知识 一、甲烷部分氧化(POM): 甲烷部分氧化(POM)制合成气的一个优势是温和的放热反应。在750~800℃下,甲烷平衡转化率可达90%以上,CO和H2的选择性高达95%,反应接触时间短(下于10-2s),可避免高温非催化部分氧化法伴生的燃烧反应,生成合成气的CO和H2摩尔比接近2,适合于甲醇生产要求。 二、甲烷转化的化学反应: 甲烷部分氧化制合成气的总反应式如下: CH4+ 1/2O2=CO+2H2+35.5kJ/mol 但实际反应过程非常复杂,而且伴有一些副反应发生,包括氧化反应、重整反应、水煤气变换反应以及积炭和消炭反应等。 ①氧化反应 CH4 + 2O2=CO2+2H2O +802kJ/mol CH4 + 3/2O2=CO+2H2O +519kJ/mol CH4 + 1/2O2= CO2+H2+561kJ/mol CH4 + 3/2O2= CO2+2H2+319kJ/mol H2+ 1/2O2= H2O +241.83kJ/mol CH4+ O2=CO+ H2O +H2+278kJ/mol ②重整反应 CH4+ H2O≒CO+3H2-206kJ/mol CH4+ CO2≒2CO+2H2-247kJ/mol ③水煤气变换反应 CO+ H2O≒CO2+H2+41.2kJ/mol ④积炭和消炭反应 CH4≒C+H2-74.9 kJ/mol 2CO≒CO2+C +172.4 kJ/mol C+ H2O≒CO +H2-131.36 kJ/mol 三、甲烷部分氧化制合成气反应的平衡常数: 甲烷部分氧化制合成气反应的平衡常数可用下面公式表示: k p= (p CO·p H22) / (p CH4·p O21/2) 式中k p——甲烷部分氧化制合成气反应的平衡常数 p CH4、p CO、p H2、p O2——分别表示甲烷、一氧化碳、氢气、氧气的平 衡分压。 对甲烷部分氧化制合成气反应CH4+ 1/2O2=CO+2H2用公式计算结果的平衡
CO2的催化转化研究进展
CO2的催化转化研究进展 摘要:能源与环境问题已经成为制约当今社会发展的两大主要问题。催化转化二氧化碳,不仅可以减少大气中的二氧化碳含量,解决温室效应带来的环境问题,而且可以提供能源燃料,具有可观的经济效益。本文综述了催化转化二氧化碳的研究进展,介绍了常用的催化材料。 关键词:二氧化碳;催化剂;转化; CO2是引起全球温室效应的气体之一, 特别是近些年来, 随着人类活动的加剧, 大气 中CO2的含量提高得更快, 进一步加剧了温室效应。通过化学转化的途径, 既能消除CO2的影响, 同时将CO2转化成有用的基本化工原料, 这将非常有益于环境和人类自身的发展。 一、CO2催化加氢制二甲醚 二甲醚是高附加值的化学产品,也是优良的新燃料,以廉价的CO2为原料制备二甲醚是一种有效利用CO2的方法,该反应过程对利用小分子碳资源、开发新能源、环保等方面都具有重要的意义,正为各国学者广泛关注,已成为绿色化学的热门课题之一。 1. CO2催化加氢合成二甲醚工艺 目前,CO2制备二甲醚主要有两种工业生产工艺,即两步法和一步法,具体来说,两步法是先合成甲醇,再由甲醇脱水得到二甲醚,将合成甲醇及合成二甲醚两个过程依次进行;一步法是由CO2加氢直接得到二甲醚。热力学上,CO2合成甲醇反应与CO2合成二甲醚反应均为分子量减少的放热反应,在相同反应条件下,对于反应过程中的甲醇浓度,CO2合成二甲醚反应比CO2合成甲醇反应低,较低的甲醇浓度促进CO2转化过程正向进行,即直接合成二甲醚反应比合成甲醇反应的热力学限制小;从设备投资上看,采用一步法将甲醇合成和甲醇脱水两个反应在同一个反应器中进行,一步法比两步法更具经济优势,一步法工艺是催化CO2合成二甲醚的发展趋势。Sosna等采用热力学方法,分析了CO2合成甲醇、合成二甲醚的工艺流程,热力学数值计算结果表明:在合成甲醇反应中的CO2单程转化率为34.02%,在一步法合成二甲醚反应中CO2单程转化率为72.72%,CO2采用一步法转化为二甲醚将获得更大的单程转化率。 2.CO2催化加氢合成二甲醚催化 CO2加氢一步法合成二甲醚是采用化学催化法对CO2进行配位活化实现的,CO2加氢一步法合成二甲醚工艺的关键点和难点是制备高效的CO2活化催化剂。目前,CO2加氢直接合成二甲醚采用由甲醇合成活性中心和甲醇脱水活性中心组成双功能催化剂。在CO2加氢直接合成二甲醚所使用的双功能复合催化剂中,甲醇合成活性组分主要为Cu基催化剂,甲醇脱水活性组分主要为HZSM-5、γ-Al2O3等固体酸。 目前的研究中,甲醇合成催化剂以Cu-Zn基催化剂为主,采用不同的助剂对Cu-Zn基甲醇合成催化剂进行改性,以提高CO2的转化率及二甲醚的选择性,采用HZSM-5分子筛进行脱水以获得二甲醚,使用该类双功能催化剂CO2转化率为15%~44%,二甲醚的选择性为40%~60%,最高达到90%。一步法合成二甲醚较合成甲醇过程有更大的热力学推动力,既能获得较高的CO2转化率,水伴随着二甲醚生成又可抑制逆水煤气反应发生,从而减少
甲烷新工业的催化转化新进展
甲烷新工业的催化转化新进展 引言 甲烷是天然气的主要成分。随着天然气在世界能源结构中的比例日趋增大,对甲烷的加工利用愈来愈受到重视。未来10年,全世界天然气消费增长率每年将保持在3. 9%左右,发展速度将超过石油、煤炭等其他能源;在全球能源结构中,天然气消费量占一次性能源消费量的比例将从现在的23. 8%提高到35. 0%。目前,我国天然气的开采和利用尚处于初级阶段,产量仅为300多亿m3 / a。甲烷作为化工原料主要限于生产合成氨、甲醇及其衍生物,其用量占天然气消耗量的5% ~7%。对此,我国提出在21世纪大力发展天然气,并与石油和煤的开发利用形成互补。可见,天然气工业将在我国21世纪的能源战略中占有举足轻重的地位。炼焦过程中产生的副产物焦炉煤气也是甲烷气体的重要来源。焦炉煤气主要由氢气和甲烷构成,同时含有少量一氧化碳、二氧化碳、氧气、氮气、硫化氢和其他烃类,对其充分利用将对环境保护、合理利用资源和实现可持续发展起到重要的作用。以3亿t/ a焦炭计算,在煤焦化过程中可副产1 350亿m3 / a焦炉煤气。其中,除部分回焦炉自身加热和用作城市煤气及发电外,有约1 /3的富余煤气,这部分焦炉煤气就成为待利用的资源。但是,由于焦炉煤气中的甲烷产生的温室效应要比二氧化碳大几十倍,其破坏臭氧层的能力也与氟利昂类似。所以,焦炉煤气的充分利用成为环境保护的需要。甲烷水合物,即天然气水合物,是甲烷的另外一个潜在来源,它是由水分子和天然气分子在一定温度和压力下形成的似冰雪状结晶化合物,又称为可燃冰。可燃冰是一种不同于常规油气的清洁环保、储量丰富的新型能源。据国际地质勘探组织估算,目前,天然气化合物含甲烷资源占全球煤、石油和天然气甲烷资源的53% ,其总能量是所有煤、天然气、石油等化石燃料能量总和的2倍~3倍。我国的甲烷水合物资源量虽未完全探明,但已经发现,我国领海及专属经济区具备甲烷水合物形成的地质构造和物源条件,具备良好的找矿前景,而且在我国南海北部等海域有存在天然气水合物矿藏的可能性。有专家认为,一旦该能源得到开采,将使人类的燃料使用延长几个世纪。甲烷水合物研究已经随着能源短缺的日益加剧,成为当代地球科学研究和能源工业发展的一大热点。综上所述,在石油资源日益减少、我国的能源消耗又日益增加的今天,充分利用甲烷资源,将甲烷直接转化成合成气和液体燃料的研究,具有巨大的经济价值和战略意义。 1甲烷催化转化制合成气的主要途径 甲烷作为化工原料生产化学品有直接转化和间接转化2种途径[ 224 ] 。直接转化是将甲烷直接转化为工业上需求的产品, 包括甲烷氧化偶联制乙烷[ 5 ] 、乙烯,甲烷选择性氧化制甲醇、甲醛等以及甲烷无氧芳构化等反应[ 628 ] 。直接转化法中,甲烷转化率和产品收率低,短期内无法实现工业化。间接转化法是将甲烷转化成合成气,进而合成氨、甲醇、乙醇等化工产品。目前,甲烷的大规模利用主要依赖于间接转化法。甲烷分子的活化是甲烷转化利用的基础,无论是何种转化,都必须经过甲烷的活化。甲烷是最简单的烷烃,甲烷分子中4个氢原子的地位完全相同,用其他原子取代其中任何1个原子,只能形成1个取代甲烷。甲烷分子具有正四面体的空间结构,这种稳定的正四面体结构决定了甲烷化学性质的不活泼性。甲烷重整就是在一定的反应条件下,通过催化作用促使甲烷的C—H键断裂,重新组合新的化学键,以利于后续工艺对甲烷的充分利用。以甲烷为原料制取合成气的传统方法为甲烷水蒸气重整法,这种方法目前已在工业上大规模应用。但甲烷水蒸气重整反应是强吸热过程,设备投资和能耗都很高,而且生产出的合成气中H2 的含量较高[ n (H2 ) /n (CO)约为3 ][ 9 ] ,不利于进一步的费托合成和甲醇合成反应的进行。利用甲烷制合成气,除了可以采用水蒸气转化及部分氧化技术以外,还可以采用二氧化碳进行重整反应。该方法可生成富含CO的合成气,既可解决蒸汽转化法中氢过剩的问题,又可实现CO2 的减排。这一堪称环境友好工程的研究动向是在
甲烷转化催化剂使用技术资料
转化催化剂使用技术 讲座 西南化工研究设计院四川天一科技股份有限公司
目录前言 Ⅰ转化催化剂的装填及蒸汽钝化 Ⅱ转化催化剂还原技术要求 Ⅲ转化催化剂运转中活性与强度损伤原因分析
前言 自七十年代后我国相继引进和建设了一大批大、中型合成氨装置,这批装置与国内其它中、小型厂相比,具有技术先进、能耗低、经济效益高的特点,如80年代初引进的以AMV工艺为主体的河南中原化肥厂总能耗可减少到29.7GJ/T氨,采用美国S·F布朗公司的深冷净化法的涪陵816厂、锦西化肥厂、合江化肥厂、新疆乌石化厂等总能耗为29.9 GJ/T氨。但与先进国家相比差距仍比较大。占我国合成氨总产量的60%的中、小型厂基本上仍使用60年代的技术。七十年代初引进的十二套大型合成氨厂能耗仍徘徊在40 GJ/T 氨,这些厂设备需要更新,还需要及正在引进部分先进技术进行节能改造。 转化炉是合成氨厂、制氢厂的关键设备,其投资高,能量损失大,转化工段能量损失占总能耗的50%以上,因而节能措施很大部分集中在一段转化炉,如降低一段炉H2O/C,回收烟气余热,将一段炉由外供热式改造为换热式,与节能工艺想适应的新型节能转化催化剂的研制等。 采用先进节能工艺对企业进行改造的同时,应充分发挥现有企业的生产能力,挖潜革新,使装置作到稳定、长周期运行,实现降低能耗及生产成本,提高氨生产能力的目标。 转化催化剂的使用性能直接影响氨厂的运转率,而且会影响转化炉管及设备的寿命,使催化剂处于最佳性能状态,是保证氨厂、制氢厂长周期稳定运转,提高经济效益的重要手段。 本讲义拟从催化剂的装填及催化剂的氧化还原等两个关键操作步骤探讨如何使催化剂处于最佳性能状态。 本讲义的各章节是独立的,并无相互关连,敬请参考阅读。
甲烷临氧催化转化制合成气研究进展
2008年第27卷第4期CHEMICAL INDUSTRY AND ENGINEERING PROGRESS ·503· 化工进展 甲烷临氧催化转化制合成气研究进展 井强山1,方林霞1,楼辉2,郑小明2 (1信阳师范学院化学化工学院,河南信阳 464000;2浙江大学催化研究所,浙江杭州 310028) 摘要;介绍了国内外甲烷临氧催化转化制合成气的研究进展,结合本课题组的研究结果及文献报道,对甲烷部分氧化、甲烷临氧二氧化碳重整、甲烷临氧水蒸气重整及甲烷-二氧化碳-水-氧气耦合三重整反应进行了阐述和分析,综述了在催化剂体系、反应机理和工艺条件等方面取得的近期研究成果。最后对甲烷临氧催化转化制合成气技术今后的研究重点及应用领域作了展望。 关键词:甲烷;合成气;部分氧化;自热重整;催化 中图分类号:TQ 426.8 文献标识码:A 文章编号:1000–6613(2008)04–0503–05 Progress of catalytic conversion of methane to syngas in the presence of oxygen JING Qiangshan1,FANG Linxia1,LOU Hui2,ZHENG Xiaoming2 (1School of Chemistry and Chemical Engineering,Xinyang Normal College,Xinyang 464000,Henan,China; 2Institute of Catalysis,Zhejiang University,Hangzhou 310028,Zhejiang,China) Abstract:In this paper,the research progress of synthesis gas preparation by catalytic conversion of methane in the presence of oxygen is summarized. The authors’ work in preparing syngas form methane in the presence of oxygen is introduced. Catalytic partial oxidation (CPO),autothermal CO2 reforming (ATR-CO2),autothermal H2O reforming (ATR-H2O) and triple-reforming of methane are reviewed. The achievements in catalyst,reaction mechanism and process conditions are also discussed. The research focus and commercial application of catalytic conversion of methane in the future are also prospected. Key words:methane;syngas;partial oxidation;autothermal reforming;catalysis 目前,工业上从天然气制合成气主要采用水蒸气重整工艺。这是一强吸热过程,投资大、能耗高,所得合成气的H2/CO比较高,适合于合成氨及制氢,而不适用于甲醇合成和费-托合成等过程。 近年来,从节约能源、降低催化剂积炭等角度出发,众多研究者在甲烷临氧催化转化领域做了大量的工作,主要的研究内容有甲烷催化部分氧化、甲烷二氧化碳临氧自热重整及甲烷水蒸气氧气混合重整等。本文作者主要综述了几种甲烷临氧催化转化制合成气方法的催化剂研究、反应器及其优缺点,并对从天然气出发制合成气路线提出自己的观点。 1 甲烷部分氧化制合成气 甲烷部分氧化(POM)制合成气是一个温和的放热反应,在750~800 ℃下,平衡转化率可达90%以上,CO和H2的选择性高达95%,生成的合成气V(H2)/V(CO)≈2,可直接用于甲醇及费-托合成等重要工业过程。与传统的蒸气重整法相比,POM在很高空速下进行,反应器体积小、效率高、能耗低,可显著降低设备投资和生产成本。进入20世纪90年代以来,这一工艺过程受到了国内外的广泛重视,研究工作十分活跃。 1.1甲烷催化部分氧化反应催化剂研究现状 POM反应所用催化剂主要是负载型金属催化 收稿日期:2007–11–22;修改稿日期:2007–12–21。 基金项目:国家自然科学基金重点资助(20433030)及河南省高校新 世纪优秀人才支持计划(2006HANCET-20)。 第一作者简介:井强山(1970—),男,博士,副教授。电话 0376–6390603;E–mail 9jqshan@https://www.360docs.net/doc/8f4126875.html,。
甲烷(CH4)的直接转化利用技术
2010年第09期甲烷(CH4)的直接转化利用技术 苗蓓蓓 大庆炼化公司档案管理中心 黑龙江大庆 163411 摘 要:目前较为成熟的技术路线是将甲烷转化为合成气,再合成甲醇或合成氨,进而开发相关的下游产品。但由于间接利用甲烷的技术路线存在投资费用高、工艺流程复杂,生产成本较高等原因,目前在工业上还并未得到大规模化应用。从原理上看,甲烷直接转化利用是最直接有效的途径。研究表明,由于甲烷的化学惰性,目前的很难在较高的甲烷转化率下获得理想的产物选择性。因此,甲烷直接转化法在工业上应用的较少,大都还处于实验室研究阶段。一旦催化技术有所突破,天然气必将成为最理想的石油替代品。 关键词:甲烷 直接转化 利用技术 一、甲烷直接制备甲醇 (1)甲烷直接部分氧化制备甲醇。甲烷直接部分氧化制备甲醇的关键技术还是催化剂,常见的催化剂目前主要是过渡金属的氧化物。例如陈立宇,杨伯伦等采用V2O5为催化剂,在发烟H2SO4中进行了甲烷液相选择性氧化的研究。V2O5催化甲烷液相部分氧化反应遵循亲电取代机理,反应为一级反应,甲烷在部分氧化反应中首先转化为硫酸甲酯,后者进一步水解得到甲醇。甲烷转化率可达54.5%,选择性45.5%。王利娟等研究了CoM004负载Mo-V-Cr-Bi氧化物催化剂上甲烷部分氧化反应,发现反应存在一转折温度,当反应温度低于此温度时,CO是主要产物,氧化产物中甲醇的选择性低于20%,而当反应温度高于此温度时,CO的选择性大大降低,而CO2的选择性大大升高,主要产物变为CO2,甲醇的选择性降为0。在甲烷首先转化生成醋酸甲酯,醋酸甲酯水解生成甲醇。在压力0.1MPa、温度267-280℃下,甲烷转化率为26.61%,目的产物选择性97.26%。 (2)甲烷和水合成甲醇。甲烷和水直接合成甲醇和H2,具有天然气资源和清洁氢能源综合开发利用的应用价值。桑丽霞,钟顺和在固定床环隙反应器中,150℃下,MoO3-TiO2/SiO2为催化剂光催化气相甲烷和水合成了目的产物甲醇和H2,甲醇选择性达87.3%。 二、甲烷制备低碳烯烃 (1)甲烷部分氧化制备烯烃。1982年美国的Union Carbide化学公司首次公开发表了甲烷催化偶联制乙烯的研究成果,该工艺是迄今为止天然气制乙烯最简捷的工艺,反应一步完成。最近LG化学公司正在进行利用天然气的主要成分甲烷生产乙烯的技术开发。这是目前世界上利用甲烷生产乙烯的首例技术尝试。甲烷氧化偶联制乙烯的技术关键在于催化剂,目前催化剂品种多达2000种以上。其中,碱金属-碱土金属、稀土金属、过渡金属氧化物和具有特定结构的复合金属氧化物等几大体系的催化剂,以及电催化、等离子催化、激光表面催化和以钙钛矿催化膜为核心的催化技术均具有较好的甲烷氧化偶联生成C2烃的反应活性。苑慧敏,张永军等综述了甲烷氧化偶联制乙烯催化剂的研究进展情况。侯思聪等采用浸渍法制备了Li-ZnO/La2O3催化剂并考察了其低温催化甲烷氧化偶联反应性能。在680℃,甲烷转化率为27.3%,C2选择性为65.2%,C2收率为17.8%的结果;在700℃,C2收率达到21.8%。王凡,郑丹星通过平衡常数法研究了500-1000℃、0.1-3.0MPa,以及进料组成中甲烷与氧的摩尔比(即n0,CH4/n0,O2)为1-10下的甲烷转化率及其他各组分收率和选择性的变化情况,在对甲烷氧化偶联制烯烃体系的热力学平衡进行分析后发现,在甲烷氧化偶联制烯烃体系中,H2、CO 的生成相对容易,C2产物(C2H6、C2H4)不容易生成。实验为甲烷氧化偶联反应器和催化剂的开发研究提供热力学依据。由于甲烷氧化偶联制乙烯反应本身受动力学控制,C2烃单程收率低,产物分离困难。目前同时能使甲烷转化率、C2选择性之和达到或接近100%的催化剂为数不多,催化剂筛选成为其实现工业化的重要阻碍。 (2)等离子体催化甲烷合成烯烃。除了传统的催化剂活化甲烷合成乙烯外,电催化、等离子催化、激光表面催化也被用于甲烷氧化偶联的催化研究中。陈韩飞等综述了等离子体活化及等离子体与催化剂协同活化甲烷转化的国内外研究进展。同时对其反应机理进行了讨论,分析了当前利用等离子体活化甲烷所存在的问题,并提出了今后的研究方向。 (3)氯甲烷路线。1988年,TaylorC.E.等人提出了甲烷经氯甲烷合成汽油产品的循环利用途径。氯甲烷转化为低碳烯烃作为天然气利用的一个全新途径,已经引起了甲烷转化研究领域的关注。甲烷首先在催化剂的作用下发生氧氯化反应得到氯甲烷,氯甲烷干燥后在催化剂上转化为汽油产品,而过程中产生的HCl可以通过循环继续参与第一步的反应形成循环过程。使用分子筛催化剂可以将氯甲烷转化为烃类产品,但产物大多数以芳烃和烷烃为主,使用镁和磷镁修饰的催化剂可以提高产物中烯烃的选择性。张大治等经过研究认为镁的修饰对催化剂酸性的影响导致了产物中低碳烯烃的增加。 (4)天然气部分氧化制乙炔。天然气部分氧化制乙炔主要采用气相氧化法,主要有德国的BASF工艺、比利时的SBA工艺和意大利的Motecatini工艺。其中,以BASF工艺为主,约占80%。BASF 工艺原料中的O2,与CH4的摩尔比为0.6,在反应炉进行复杂的气相反应,主要反应通过部分甲烷进行部分氧化提供热量,剩余甲烷被加热到1500℃后裂解缩合为乙炔。 三、甲烷制备芳烃 (1)甲烷部分氧化制备芳烃。上个世纪80年代,Shepelev等对甲烷催化氧化制芳烃技术进行了研究,结果表明,在氧化条件下,甲烷合成芳烃的反应很难控制,甲烷的转化率很低,芳烃选择性和收率也很低,在经济上不具备开发前景。舒玉瑛等发现,不同方法制备的Mo/H-ZSM-5催化剂上甲烷的芳构化反应,对甲烷制备芳烃反应有较大的影响。 (2)甲烷无氧脱氢制备芳烃。从热力学角度来讲,甲烷直接转化为芳烃要比直接转化为乙烷和乙烯更为有利。而且,在无氧条件下也不生成CO和CO2。自1993年大连化学物理研究所首先报道了在无氧和连续流动的反应条件下,甲烷在Mo/HZSM-5催化剂上直接转化为芳烃以来,甲烷无氧芳构化已经成为甲烷直接催化转化研究中的一个重要分支,是目前甲烷直接转化的主要研究内容。魏飞等综述了利用甲烷直接脱氢制备芳烃的催化剂方面的研究情况,此外,郑海涛等人还研究了甲烷和丙烷混合气体在不同催化剂上的无
甲烷转化
K.M.Shen for helpful discussions and communications.Experimental studies were supported by the Center for Emergent Superconductivity,an Energy Frontier Research Center,headquartered at Brookhaven National Laboratory (BNL)and funded by the U.S.Department of Energy under grant DE-2009-BNL-PM015,as well as by a Grant-in-Aid for Scientific Research from the Ministry of Science and Education (Japan)and the Global Centers of Excellence Program for Japan Society for the Promotion of Science.C.K.K.acknowledges support from the FlucTeam program at BNL under contract DE-AC02-98CH10886.J.L.acknowledges support from the Institute for Basic Science,Korea.I.A.F.acknowledges support from Funda??o para a Ciência e a Tecnologia,Portugal,under fellowship number SFRH/BD/60952/2009.S.M.acknowledges support from NSF grant DMR-1120296to the Cornell Center for Materials Research.Theoretical studies at Cornell University were supported by NSF grant DMR-1120296to Cornell Center for Materials Research and by NSF grant DMR-0955822.The original data are archived by Davis Group,BNL,and Cornell University. Supplementary Materials https://www.360docs.net/doc/8f4126875.html,/content/344/6184/612/suppl/DC1Materials and Methods Supplementary Text Figs.S1to S9 References (42–45)Movies S1and S2 21November 2013;accepted 20March 201410.1126/science.1248783 Direct,Nonoxidative Conversion of Methane to Ethylene,Aromatics,and Hydrogen Xiaoguang Guo,1Guangzong Fang,1Gang Li,2,3Hao Ma,1Hongjun Fan,2Liang Yu,1Chao Ma,4Xing Wu,5Dehui Deng,1Mingming Wei,1Dali Tan,1Rui Si,6Shuo Zhang,6Jianqi Li,4Litao Sun,5Zichao Tang,2Xiulian Pan,1Xinhe Bao 1* The efficient use of natural gas will require catalysts that can activate the first C –H bond of methane while suppressing complete dehydrogenation and avoiding overoxidation.We report that single iron sites embedded in a silica matrix enable direct,nonoxidative conversion of methane,exclusively to ethylene and aromatics.The reaction is initiated by catalytic generation of methyl radicals,followed by a series of gas-phase reactions.The absence of adjacent iron sites prevents catalytic C-C coupling,further oligomerization,and hence,coke deposition.At 1363kelvin,methane conversion reached a maximum at 48.1%and ethylene selectivity peaked at 48.4%,whereas the total hydrocarbon selectivity exceeded 99%,representing an atom-economical transformation process of methane.The lattice-confined single iron sites delivered stable performance,with no deactivation observed during a 60-hour test.T he challenge of converting natural gas into transportable fuels and chemicals (1)has been spurred by several emerging indus-trial trends,including rapidly rising demand for H 2(for upgrading lower-quality oils)and a global shortage of aromatics caused by shifting refinery targets toward gasoline.Light olefins,which are key chemical feedstocks,are currently made from methanol,which itself is made through multistage catalytic transformations via syngas (a mixture of H 2and CO)(2,3),although there is also ongoing research to convert syngas directly to light olefins (4,5).However,in all such approaches,either CO or H 2is needed to remove oxygen from CO,result-ing in a carbon-atom utilization efficiency below 50%.Despite their low efficiency,high capital and production costs,and enormous CO 2emissions, syngas routes dominate current and near-term in-dustrial practices for natural gas conversion (6,7).Direct conversion of CH 4is potentially more economical and environmentally friendly but is challenging because CH 4exhibits high C –H bond strength (434kJ/mol),negligible electron affinity,large ionization energy,and low polarizability (8).In the pioneering work of Keller and Bhasin in the early 1980s,CH 4was activated with the assistance of oxygen (9).This finding initiated a worldwide research surge to explore the high-temperature (>1073K)oxidative coupling of methane (OCM)to C 2hydrocarbons (10,11).Hundreds of catalytic materials have since been synthesized and tested,principally during the 1990s,as well as in recent years.Unfortunately,the presence of O 2leads irreversibly to overoxidation,resulting in a large amount of the thermodynamically stable end-products CO 2and H 2O.Thus,the carbon utili-zation efficiency of OCM remains relatively low (12,13).Slow progress in discovering new cata-lysts to circumvent this problem has hindered further development,and no economically viable process has been put into practice so far. In a recent report,elemental sulfur was used as a softer oxidant than O 2(14):For a 5%CH 4/Ar mixture at 1323K,the best catalyst,PdS/ZrO 2,gave a CH 4conversion of ~16%and ethylene selectivity near 20%,albeit at the expense of the by-products CS 2and H 2S (14).In contrast,the bifunctional catalysts based on Mo/zeolites cata-lyze CH 4conversion to aromatics (benzene and naphthalene)nonoxidatively,thereby avoiding CO 2formation (15–18).CH 4is activated on the metal sites forming CH x species,which dimerize to C 2H y .Subsequent oligomerization on the acidic sites located inside the zeolite pores yields ben-zene and naphthalene,as well as copious amounts of coke (19–21).Commercial prospects for this process are further hampered by the instability of zeolites at the very high reaction temperatures.To achieve direct conversion of CH 4efficient-ly,the challenges lie in cleaving the first C –H bond while suppressing further catalytic dehy-drogenation,avoiding both CO 2generation and coke deposition.We report that these conditions can be met using lattice-confined single iron sites embedded in a silica matrix.These sites activate CH 4in the absence of oxidants,generating methyl radicals,which desorb from the catalyst surface and then undergo a series of gas-phase reactions to yield ethylene,benzene,and naphthalene as the only products (with ethylene dominating at short space-times for a selectivity of ~52.7%at 1293K).A methane conversion as high as 48.1%is achieved at 1363K. The catalysts were obtained by fusing ferrous metasilicate with SiO 2at 1973K in air and from commercial quartz,followed by leaching with aqueous HNO 3and drying at 353K (22).The resulting catalyst was designated 0.5%Fe?SiO 2(?denotes confinement and here represents a cat-alyst characterized by the lattice-confined single iron sites embedded within a silica matrix).It con-tained 0.5weight percent (wt %)Fe and had a Brunauer –Emmett –Teller surface area of <1m 2/g.The catalyst was activated in a fixed-bed micro-reactor in the reaction atmosphere [90volume percent (vol %)CH 4/N 2]at 1173K.The efflu-ent was analyzed by online gas chromatography (GC).At 1223K,CH 4conversion was 8.1%(Fig.1A)and increased with temperature,exceeding 48.1%at 1363K (Fig.1B).Only ethylene,ben-zene,and naphthalene were produced;neither coke nor CO 2was detected,despite the relative-ly high reaction temperature.A single-pass yield of 48%hydrocarbons is achieved at 1363K and 21.4liters per gram of catalyst (gcat)per hour.Selectivities vary from 40.9to 52.1%for ethylene,21.0to 29.1for benzene,and 23.6to 38.2%for naphthalene,over the investigated temperature range (1223to 1363K). By comparison,a blank experiment (an empty reactor with no catalyst)under the same conditions showed a CH 4conversion of only 2.5%,and 95%of the product was coke (Fig.1A).A test with unmodified SiO 2as the catalyst yielded virtually 1 State Key Laboratory of Catalysis,Dalian Institute of Chemical Physics,Chinese Academy of Sciences,Dalian 116023,Peo-ple ’s Republic of China.2State Key Laboratory of Molecular Reaction Dynamics,Dalian Institute of Chemical Physics,Chinese Academy of Sciences,Dalian 116023,People ’s Republic of China.3State Key Laboratory of Fine Chemicals,Institute of Coal Chemical Engineering,School of Chemical Engineering,Dalian University of Technology,Dalian 116012,People ’s Republic of China.4Beijing National Laboratory for Condensed Matter Physics,Institute of Physics,Chinese Academy of Sciences,Beijing 100190,People ’s Republic of China.5Nano-Pico Center,Key Laboratory of Micro-Electro-Mechanical System (MEMS)of Ministry of Education,Southeast University,Nanjing 210096,People ’s Republic of China.6Shanghai Synchrotron Radiation Facility,Shanghai Institute of Applied Physics,Chinese Academy of Sciences,Shanghai 201204,People ’s Republic of China.*Corresponding author.E-mail:xhbao@https://www.360docs.net/doc/8f4126875.html, 9MAY 2014VOL 344 SCIENCE https://www.360docs.net/doc/8f4126875.html, 616REPORTS o n J u l y 12, 2016 h t t p ://s c i e n c e .s c i e n c e m a g .o r g /D o w n l o a d e d f r o m